Michigan State University’s Andrew Christlieb is leading a massive U.S. Department of Energy project to help deliver on the not-yet-realized promise of nuclear fusion. That promise? To create an unmatched source of affordable and sustainable energy.
Christlieb, an MSU Foundation Professor in the College of Natural Science, is now the director of a Mathematical Multifaceted Integrated Capability Center, or MMICC, supported by $15 million in funding from the DOE. He is joined by researchers at eight other universities and national labs across the country. Together, they’re developing new mathematical and computational tools to better model the physics needed to understand, control and sustain fusion.
The MSU-led center is one of four new MMICCs announced by the DOE.
“MMICCs enable applied mathematics researchers, working in large, collaborative teams, to take a broader view of a problem,” said Barbara Helland, DOE associate director of science for the Advanced Scientific Computing Research program, in a recent news release. “As a result of this holistic view, the researchers devise solutions by building fundamental, multidisciplinary mathematical capabilities considering existing and emerging computing capabilities.”
“We’re going to be pushing the boundaries of what can be done mathematically and computationally,” says Christlieb, who is a professor in the Department of Mathematics and the Department of Computational Mathematics, Science and Engineering.
In addition to MSU’s contingent of experts, the team includes collaborators from Lawrence Livermore National Laboratory, Los Alamos National Laboratory, Oak Ridge National Laboratory, Sandia National Laboratories, the University of Colorado-Boulder, the University of Delaware, the University of Massachusetts-Dartmouth and the University of Washington.
“We’re asking ourselves how do we engage with things like machine learning? How do we engage with bigger, more powerful computers? How do we engage with new mathematical algorithms?” Christlieb says. “We have this lofty goal of taking a bird’s-eye view, looking down on all these different pieces and understanding how they fit together to solve big problems.”
As one might imagine, simultaneously pushing the boundaries of math, computation and nuclear fusion is full of complex technical challenges.
Fortunately, understanding the bigger picture of what this MMICC can deliver does not require advanced mathematics or sophisticated algorithms. In fact, whether they know it or not, everyone on this planet already has an appreciation for the impact of fusion.
Harnessing the power of the sun
What Christlieb and his colleagues are trying to do is help power the planet using the same science that powers the sun: nuclear fusion. To do this, the team is working to bring new computational tools and techniques to bear on understanding the plasmas needed to sustain fusion.
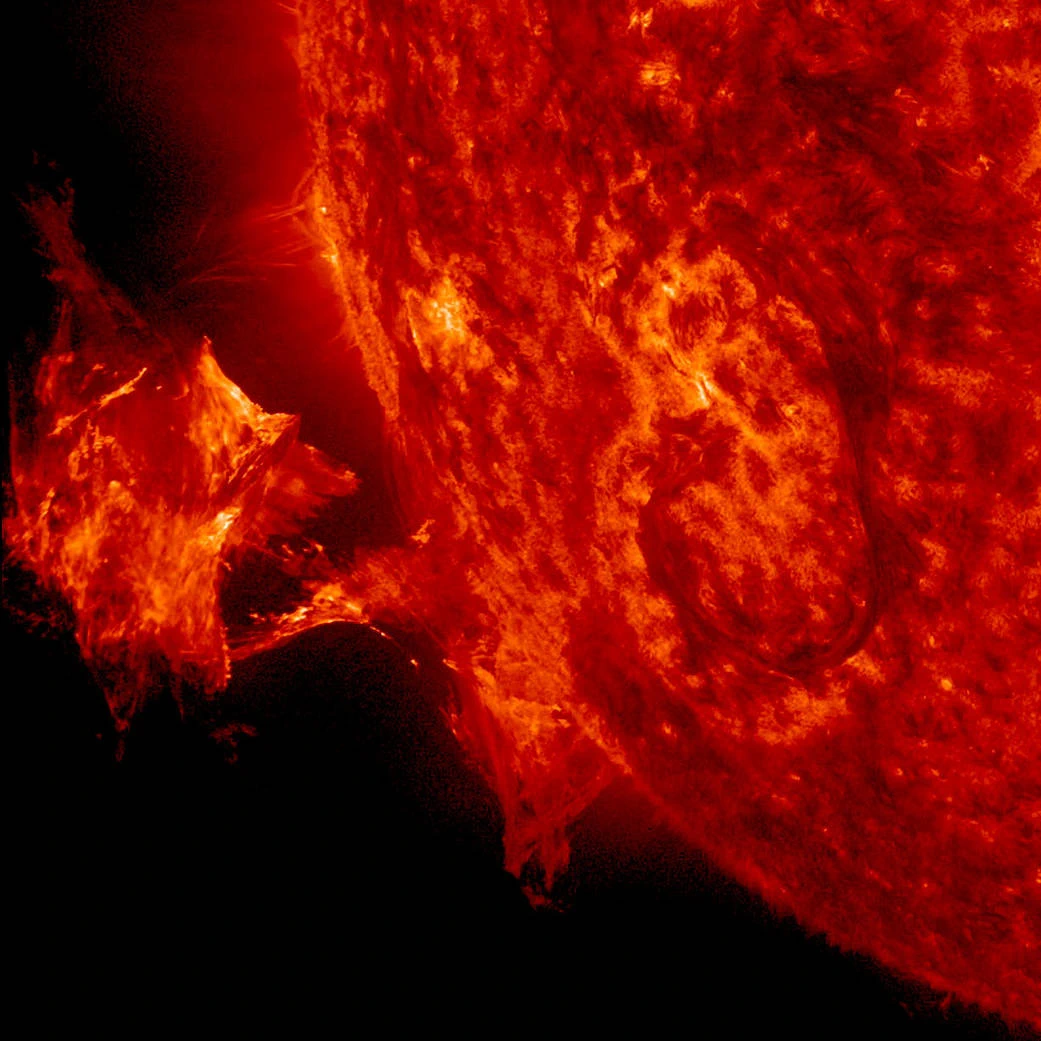
The sun itself is a ball of mostly plasma, a cloud of matter so hot that its atoms have started shedding the electron cloaks that surround their cores, or nuclei. Within the burning plasma, nuclei can collide and fuse together.
These fusion events release a ton of energy, which keeps the plasma burning and empowers subsequent fusion reactions. The sun and its fusion are thus self-sustaining, like an enormous fire that stokes itself and showers the solar system with light and heat.
For decades, humanity has been trying to replicate what the sun does at a much smaller scale to help meet the world’s energy needs. Scientists achieved their first fusion reaction in the 1930s.
In the nine decades since, however, researchers have yet to ignite self-sustaining reactions that reliably generate more energy than they consume. One of the hurdles has been understanding the plasmas involved well enough to control and optimize them.
By the early 2000s, plasma and fusion researchers had seen enough dead ends and false starts that many began to doubt people would ever realize this untapped potential. That included Christlieb’s doctoral adviser.
“He advised me to not work on fusion. At the time, it seemed that the plasma community had lost its way, and fusion just wasn’t going to produce energy,” Christlieb says. But things started to change as the 21st century progressed, and researchers brought fresh insights to the field.
“Technologies we thought were dead all of a sudden became more interesting,” Christlieb says.
With that, there’s a renewed sense of optimism and urgency about fusion. In March of this year, the White House hosted a summit focused on accelerating the development of fusion energy. There are also more than a dozen start-up companies in the U.S. and Canada currently developing fusion-related technology.
“Some of these are really exciting,” Christlieb says. “I really believe fusion energy is on the horizon.”
Questions about plasmas, however, still loom large. That’s why the DOE is investing $15 million in a center that will help answer them.
“It really behooves us to use our resources wisely and enable things that weren’t possible before,” says Luis Chacon, co-director of the new MMICC, which the team has named the Center for Hierarchical and Robust Modeling of Non-Equilibrium Transport, or CHaRMNET. Chacon is a leading expert in modeling plasmas for fusion in the Theoretical Division at Los Alamos National Laboratory.
“You have to be ambitious. What we’ve proposed is a real departure from the current state-of-the-art and, any time you do that, it’s like jumping from a plane,” he says. “You hope the parachute opens, and you try to land in a good place.”
Teamwork makes the plasma dream work
With that analogy in mind, it’s easy to understand Chacon’s feelings as the project gets going.
“It’s elation mixed with adrenaline and a little bit of ‘What the heck am I getting into?’” he says, which is a little surprising to hear, given the calm confidence with which he says it.
Partially, that confidence comes from experience. Jumping into the unknown is what you do as a scientist, he says. But another crucial factor is the team.
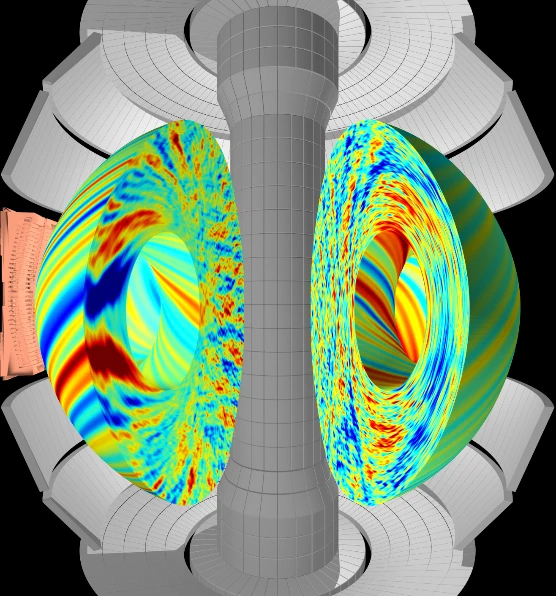
“The sheer quality of this team is massive. We found the highest quality people we could for this project,” Chacon says. Including Christlieb and Chacon, the team includes 20 investigators and their teams.
Joining the team from academia are Yingda Cheng, Huan Lei and Brian O’Shea from MSU; David Bortz and Stephen Becker from the University of Colorado-Boulder; Jingmei Qiu at the University of Delaware; Sigal Gottlieb from the University of Massachusetts-Dartmouth; and Jingwei Hu from the University of Washington.
The team’s national lab cohort includes Joshua Burby and Qi Tang from Los Alamos National Laboratory; Youngsoo Choi, Terry Haut, Jeffrey Hittinger, Lee Ricketson and Paul Tranquilli from Lawrence Livermore National Laboratory; Cory Hauck from Oak Ridge National Laboratory; and John Jakeman and Timothy Wildey from Sandia National Laboratories.
“This is the largest and highest-profile project that I’ve ever been a part of,” says Wildey of Sandia, who is an expert in machine learning and uncertainty quantification. He’s also a Spartan alumnus who graduated as part of the class of 2001 with a bachelor’s degree in mathematics.
“Just with the size and dispersity — we’re spread across nine different institutions — there will be challenges. But they’re challenges we’re looking forward to,” Wildey says. “We have an absolutely amazing team. That’s why we were successful in getting this opportunity and why I believe we’ll be successful with our project.”
Which begs the question, what does success look like and what makes achieving it so challenging?
Taming the curse of dimensionality
Wildey has a concise way of summing up the research team’s goals. The center was created to provide end-to-end computational approaches that enable fusion researchers to simulate plasmas quickly, completely and reliably enough that they can use the results to make important decisions in real time.